"It's so simple to make brain organoids. There is even a kit you can buy that has everything in it"
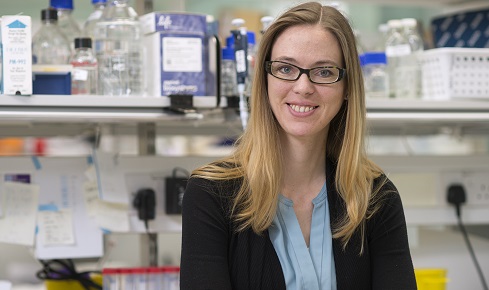
Developmental biologist Dr Madeline Lancaster discusses how she almost accidentally created cerebral organoids, and the fascinating insights they are revealing about what makes the human brain special
September 28th 2022
Dr Madeline Lancaster is group leader at the Medical Research Council's Laboratory of Molecular Biology in Cambridge. Her lab uses cerebral organoids — small aggregates of stem cells that have developed into 3D brain tissue — to study brain size, diseases of the brain, and human evolution —in a dish.
A mixture of serendipity and curiosity led Lancaster to develop cerebral organoids while culturing stem cells at the Institute of Molecular Biotechnology in Vienna around 2012. The organoids have quickly proven to be useful in modelling early brain development and neurodevelopmental disorders, and have prompted much debate over the ethics of growing ever more complex and mature 'mini-brains'.
You first created cerebral organoids almost by accident. Can you tell me a bit about how you first went from growing flat brain cell cultures to making three-dimensional ones?
There was a lot of serendipity, although I will take some credit for seeing something interesting and deciding to follow it. I was trying to coat plates with the stuff stem cell biologists use to get their cells to stick, and it wasn't working. Instead of sticking, they formed little 3D aggregates. I started playing with gel, still trying to get them to stick, but they embedded into the gel, then they really formed these amazing aggregations.
I am trained as a developmental biologist, so I wasn't thinking like a stem cell biologist. Their goals were often to make these really pure preparations of cells that could be used to test treatments – they don't want to have any contaminating cell types in there. I was doing the complete opposite; I didn't want simplicity, I wanted complexity and intrinsic self-organisation. I wanted the cells to just do what they want.
Coming from a developmental biology perspective was what led me to investigate what I’d made further. I spoke to at least one stem cell biologist who said he’d had the same results as me and had just thrown them out.
Is there something about growing brain cells that made this happy accident more likely than someone else working on a different tissue?
It's not so much about the cells bunching together but the fact that brain is very easy to make. You may think that brain would be the most difficult thing, but in fact the first decision that an embryonic stem cell is going to make is, ‘do I make brain, or everything else’? In order to make brain, all you really have to do is avoid cells deciding 'everything else'. To get different tissue like heart you have to put on certain sera, but if you keep the cells in a minimal media, all that really survives is the brain lineage.
So brain organoids are actually a surprisingly simple system to set up and start studying then?
So simple. The only thing you need is a little shaker, which everybody has in a lab, and you just stick that in an incubator. There is even a kit you can buy from a company and you just follow the protocol. The hard part is not making them, it’s knowing what to do with them and how to analyse them.
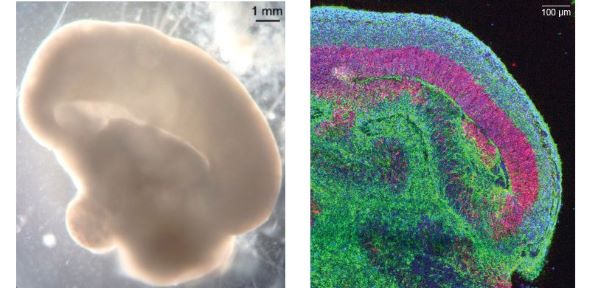
In terms of analysis, then – what are you trying to find out with your cerebral organoids?
Most of what we're interested in is fundamental, just gaining a better understanding of human brain development. The beauty of organoids is that we can make them from stem cells from any animal, as long as we have their stem cells. We’ve been doing a lot of work with cells from chimpanzees and gorillas. Nobody actually knows what a foetal brain of a chimpanzee is like, all we have is ultrasound images. Organoids suddenly give us this ability to track how human and chimp brains differ at the earliest stages of development.
When we make human, chimpanzee and gorilla organoids, what's beautiful is you can immediately see the size difference, there's this intrinsic determination of size. Human organoids are about two times bigger than the ones from the chimpanzee and the gorilla. They start to diverge already when there aren’t even any neurons yet, just this primordial tissue that's expanding and setting up a foundational stem cell pool. Eventually these cells change shape and start to make neurons. But when they start making neurons it means they can't make as many of themselves.
We found that the change in shape is happening a little bit later in humans. So that means the foundational cells are dividing faster for a longer amount of time. That means they have double the number of these founder stem cells, and then if everything else happens at the same rate, then you just get a two fold increase in everything that those cells will make. All the different neuron types, the glia they're going to make, everything is doubled.
If you look at adult humans compared to chimpanzees, you find exactly that – that all of the neuron types of the cortex are expanded equally, it's not just one particular cell type that's increased. Most people would have thought our species would be very similar early in development and the differences would only come later. It's surprising to see the difference between us and our closets living relatives is actually something so fundamental and early.
You’ve used organoids to study microcephaly, also an issue of the size of the brain.
Yes, we modelled a genetic form of microcephaly, because mouse models have not been great at modelling that. When we made the organoids we saw this really striking size difference. It’s a different mechanism, after the stem cells have started making neurons, when they're doing what's called an asymmetric cell division. They make one differentiated cell, for example a neuron, and one more of themselves.
In the patient-derived organoids, instead of these nice self-renewing divisions, the stem cells make two neurons. Then they're gone. So initially, the organoids actually have more neurons. But pretty soon there's no more stem cells to make more, so overall the brain ends up being a lot smaller.
Can you tell me a little bit about some of the methods being developed to help organoids grow for longer and get bigger?
The good thing about working with the brain is that the cortex, in particular, is not actually vascularized until roughly the transition to the second trimester of pregnancy. So all these early events we're looking at don't really need vascularisation. It starts to become a problem as you get into later stages, when you have huge numbers of neurons being made.
Some groups have been transplanting human organoids into mice, to get some vascularization of the organoids and longer term survival before taking them out to study them. That is a very involved approach. My lab takes a different approach similar to developmental biologists taking very thin slices of brain from embryos and culturing them. Those slices are thin enough that just through diffusion they'll be able to get their nutrients. You can keep neurons alive and happy like that for a long time.
We actually don't know how long we can keep them alive – we had some that were about two and a half years old, and then COVID struck and we had trouble getting access to the building to feed them.
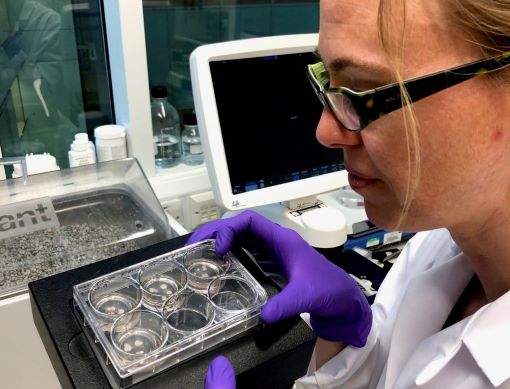
Of course the immediate question when you hear about two year old organoids in dishes is around sentience. At what point would you start to worry about that?
I've had a lot of conversations with ethicists about whether these things could become conscious or feel pain at some point. Of course there are no pain sensors in the brain, I mean pain in the sense of suffering. To answer that we first have to ask what we know is required to make a fully sentient being. We have a lot of information on that from human beings and the rest of the animal kingdom.
Sentience, depending on who you talk to, is probably more quantitative rather than qualitative. You can see some degree of sentience in a mouse, probably not so much in a fly – and it has to do with things like the organisation of the brain, the sheer size and the number of neurons, and the long range networks that form and integrate information from lots of different regions of the brain. That's increasingly recognised as being at the heart of consciousness.
So, in organoids, even if you could vascularize them and keep them fully 3D for longer, there's still so many things they would lack. They're tiny, they're not organised in the right way, they don't have these long range connections between regions, and they don't have any input or output – they can't interact with their environment at all.
I don't doubt that they are nowhere near any animal’s level of sentience. But what work has been done to understand what is going on in these organoids?
There is certainly activity, but that's what neurons do. If you culture a single neuron in a dish all by itself, it wants to be active so badly that it will wrap its axon around itself, and start connecting to itself and firing. But that doesn't mean that it's thinking.
Measuring consciousness even in an actual conscious being is tricky. And a lot of those measurements are not things that we could do on organoids because they are not organised and have no senses. For example perturbational complexity, done on brain dead or potentially brain dead people to test if they are actually brain dead: you stimulate a part of the brain, and then you look for long-range activity that's happening elsewhere in the brain that is not random but is coordinated activity in response. In organoids we just don't have any long range connections, so you couldn’t apply such a measurement.
If you could create some brain tissue with some degree of sentience or consciousness, would you?
I don't see the value of doing that. I'm really not concerned about this as organoid science stands right now, but I am a human being with empathy, and I wouldn't like to be a conscious brain in a dish. So no, I don't want to create something like that and I don't think anybody in the field is trying to do that.
I do think as a community we should pre-emptively think about these ethical issues, and ask ‘what would be a step over the line?’ I think if we could have a checklist of all the things that we know are required for consciousness, we know we have crossed the line if we ever end up with tissue that has all of those things.
Obviously, the language that we use to describe this work is really important too, and the term ‘mini-brains’ gets used a lot. Do you wish it wasn’t?
As a community we have really moved away from that term. It was kind of fun in the beginning, because all these different organoids were 'mini' guts, mini-lungs, mini-kidneys. But the brain is special, it’s not like the kidney or gut. When you start saying 'mini brain' it has connotations that can be disturbing to the public.
One of the reasons why I had these made [Lancaster shows me some tiny cerebral organoids, mounted in clear resin] is so I could take them with me whenever I do any sort of public outreach to show people. When you see them, I think most of the time fears are allayed. It's hard to imagine that this thing is really thinking.
Lancaster and her colleagues have been using cerebral organoids to understand brain cells’ susceptibility to SARS-CoV-2. Lancaster has found that cells within a region called the choroid plexus are particularly susceptible to infection – and that this region could be a weak spot that allows the virus to enter the brain. “The choroid plexus is special – it is a brain region that people overlooked for a very long time because it ‘just’ makes cerebrospinal fluid (CSF) and I suppose was seen as boring,” says Lancaster. “But not only is it making this vital fluid, it's a very thin barrier. Essentially on one side you've got the brain and the CSF that it's producing, on the other side, you've got all the blood which it's using to filter and to make the fluid. Elsewhere the blood brain barrier is very tight – there are lots many cells that contribute to it and it has multiple levels of protection. Whereas a virus could enter the brain very easily through the CSF.”
The work correlates with post-mortem studies which show high levels of virus in the choroid plexus of patients who have had COVID. Lancaster believes that once this region is infected, the brain’s immune response could be what is causing the variety of neurological symptoms seen in COVID and long COVID. “My theory is that it's not just about what the virus does in terms of getting to the brain, but the side effect of having broken this barrier, with free flowing cytokines and immune cells. You don't want T cells just flowing into the brain, because you get neuroinflammation, which is what is being seen on patient MRIs.”
What's the next step or direction for cerebral organoids?
Probably we're going to see more labs making different brain regions, and connecting them up. There are what some people are calling assembloids, a contraction of assembled organoids.
Then there are studies showing that with organoids, you can actually produce cells that are more like the real thing than if you culture them in a traditional cell line. So I think we'll see people using organoids in order to make the cells they want for disease modelling and then potentially even translation to the clinic. Disease modelling is probably the most widespread application of organoids. We did this with microcephaly, but others are doing this with autism spectrum disorder, schizophrenia, other neurodevelopmental abnormalities like dysplasias in the brain, epilepsy. Again, human brains behave in ways that just can’t be modelled in animal models.
Where did you get your interest in the brain from? I understand your mother is a psychiatrist – was that an influence on you?
Definitely. She worked for the State of Utah, where I grew up. I remember sometimes I had to sit in the waiting room while I waited for her to finish work, and some of the people that were sitting there were clearly having a psychotic breakdown. While I was never scared, it was always just really sad, and I think made me wonder about our amazing capabilities that make us uniquely susceptible to these really devastating conditions. Something like schizophrenia is actually not very severe in terms of physiology – you can't see it on an MRI. The mechanism is probably actually quite subtle. But because we have such complex cognition, if something is just slightly off it can end up being so traumatic.
Dr Madeline Lancaster is a development biologist and a group Leader in the Cell Biology Division of the Medical Research Council (MRC) Laboratory of Molecular Biology, part of the Cambridge Biomedical Campus in Cambridge, UK.