Waste opportunity
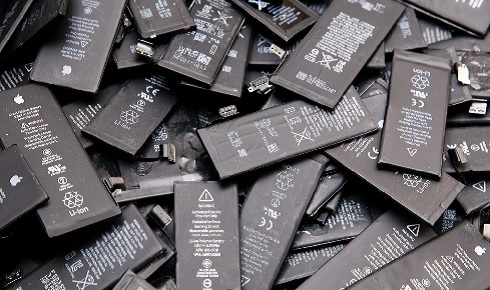
Could metalotollerant bacteria help produce valuable metal nanoparticles from the world’s spent batteries? Giovanni Maddalena, Professor Louise Horsfall FRSB and Dr Virginia Echavarri-Bravo explain
28th September 2020
Lithium-ion batteries power our mobile phones, laptops and, increasingly, electric vehicles. These batteries, produced in huge numbers, are currently either disposed of or recycled unsustainably[1]. Disposal leads to the leaching of toxic metals into the soil and ground water, while recycling requires immense amounts of energy and produces polluting chemicals. Since the recycling processes are relatively inefficient, there is often a loss of metals too.
The UK Government has committed to banning the sale of petrol, diesel and hybrid cars by 2035, meaning the demand for electric cars and lithium-ion batteries is set to rise further. A variety of metals can be used to build a lithium-ion battery, including aluminium, cobalt, copper, lithium, manganese and nickel. As electrification increases in the UK and globally, the demand for these metals will only increase, putting supply at risk[1]. There are also concerns about the environmental and ethical effect of metal mining, such as in the Democratic Republic of Congo, where cobalt production is closely tied to unethical child labour.
Recognising the importance of these issues and the significance of the automotive industry to the UK economy, the Government is supporting advanced battery research. ReLiB, a project set up by the Faraday Institution, is looking specifically at the issue of recycling lithium-ion batteries[2]. The aim of the project is to recover as close to 100% of the materials as possible and one solution being examined is the use of biology in metal recovery. From ground coffee and olive pits to lignin and plastic, biology can be used to upcycle waste, with unwanted byproducts and materials turned into a more valuable commodity. Scientists are now looking at using metallotolerant bacteria to recover the metals from spent batteries, with the obvious benefit that such microbes would be cheap, easy to grow and require little energy input to perform chemical reactions.
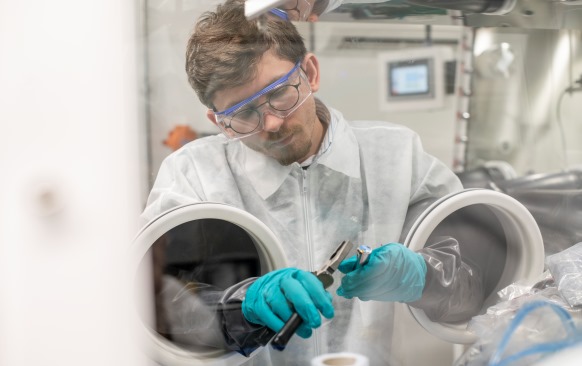
Before any waste can be biologically processed into metallic nanoparticles, the metals must be in a soluble form. Acidic solvents are used to turn the battery material into a metal ion soup, or leachate. To maintain an eco-friendly process, biodegradable solvents can be used. Citric acid, for example, is safely broken down by many organisms found in soil. The battery leachate can then be treated with nanoparticle-producing bacteria.
Some bacteria can recover metals through the synthesis of nanoparticles – metallic particles with a dimension less than 100nm. This can be done synthetically using physical and chemical processes, but these are energy intensive and often generate toxic wastes. The biogenic metallic nanoparticles produced can be a valuable product. Their surface area to volume ratio is greater compared with larger particles[3], creating interesting and useful properties. And so the biological recovery of these functional materials can add economic as well as environmental value to the recycling process.
Going to extremes
The battery leachate contains high concentrations of metal ions that would be toxic to most organisms. This means that this form of bioremediation requires so-called ‘metallotolerant’ bacteria, a branch of extremophiles that are specialised in surviving in rich metal ion solutions – for example, Cupriavidus metallidurans CH34, which thrives in the soil surrounding metal-processing plants. Two megaplasmids found in the CH34 species, pMOL28 and pMOL30, code for proteins that confer resistance to a wide range of metals: cadmium, cobalt, copper, chromium, gold, lead, mercury, nickel, silver and zinc[4]. Other parts of the genome encode for proteins that defend against arsenic.
Geobacter and Shewanella are two well-characterised exoelectrogens – microorganisms capable of transferring electrons extracellularly[5] and hypothesised to be key players in the geochemical cycling of metals. They can extract electrons from metals in the environment, but can also carry them back, producing nanoparticles.
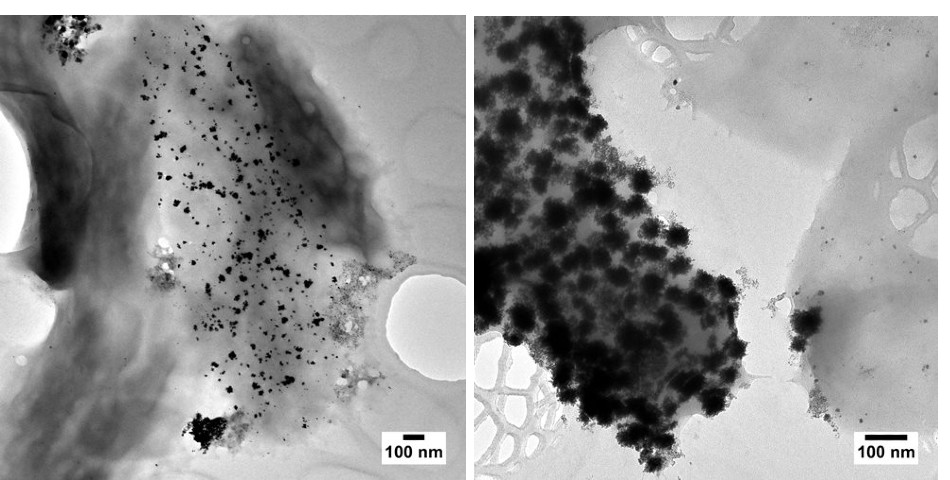
The synthesis of metallic nanoparticles in exoelectrogens has been attributed to various components of the electron transport chain at the heart of respiration. Cytochromes, or iron-containing enzymes, are believed to be one of the main acting proteins. They can use metal ions as electron acceptors or donors[6]. Sulphur-reducing microbes are also capable of producing metallic nanoparticles[7]. These microorganisms excrete hydrogen sulphide, a strong reducing agent and chemical commonly used in the chemical synthesis of nanoparticles and bio-based metal-recovery systems.
So what actually causes the nanoparticles to form? Our current understanding is that biomolecules such as lipids and peptides can play an important role[8]. They can chelate, reduce and stabilise metals, helping hold metal ions in place and stabilise the newly formed metal cluster. Redox reactions – where electrons are transferred – are central to the metals’ precipitation, with reducing and oxidising agents allowing for the flow of charge between molecules. This exchange can turn the metal ions into a less-soluble form, creating a nanoparticle.
Nanoparticles can be found extracellularly, intracellularly or membrane-bound, and recovering the particles from each synthesis method presents its own challenges. The last two provide nanoparticles that are easy to recover in association with their microbial host, but the nanoparticles themselves then need to be separated from the cell. On the other hand extracellular nanoparticles are simpler to synthesise, but are tedious to concentrate from the cells’ supernatant. Upcycling spent lithium-ion batteries into metallic nanoparticles through a microbial process is a promising alternative to the accumulation of mountains of battery waste. However, one of the greatest challenges – as is the case with most biotechnological processes – is to replicate the process consistently and on a large scale. Recent advances in genetic engineering are enabling us to overcome some of these hurdles.
Escherichia coli is a power tool for synthetic biologists as it is a robust, fast-growing and well-characterised organism. It has been engineered to produce a large variety of metal nanoparticles – including some that are found in lithium-ion batteries, such as cobalt, manganese and nickel[8] – by adding genes encoding for specialised proteins such as metallothionein and phytochelatin synthase. Metallothioneins are a family of proteins that can bind and reduce heavy metals. They form a defence mechanism found in many organisms, including plants and mammals. Similarly, phytochelatin synthase is an enzyme that produces phytochelatins – sulphur-rich metal binding peptides. By expressing these two proteins E. coli becomes more resistant to a whole host of metal ions and converts them into nanoparticles.
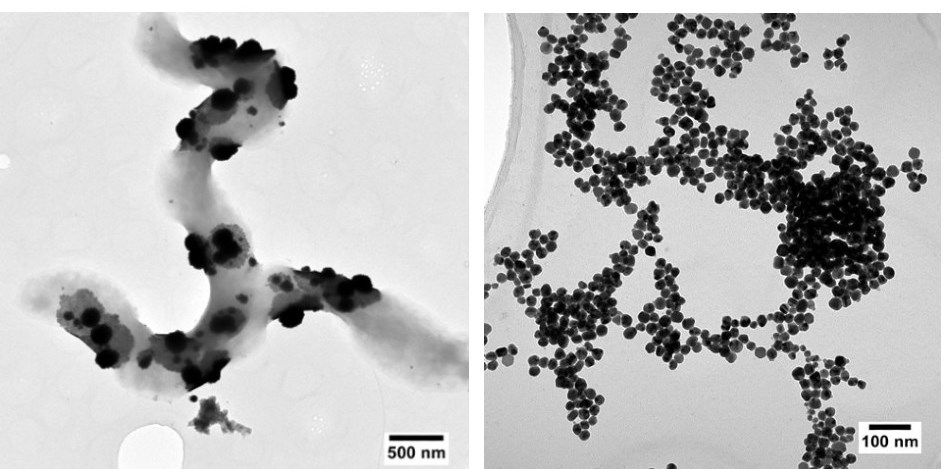
Although E. coli is often used as a workhorse for synthetic biology, it is not a model organism in the context of working with metals. Its ability to form nanoparticles is much more limited compared with that of some extremophiles. Sulphur-reducing bacteria, for example, have innate tolerance to toxic metals and lower temperature requirements for precipitating them.
These non-model organisms present us with novel pathways that we can exploit. For example, a sulphur-reducing bacteria of the Desulfovibrio species was found to express more NiFe hydrogenase in the presence of platinum ions than it normally would[9]. The bacterium was then engineered to overexpress this protein further. When exposed to platinum ions it would produce larger nanoparticles than the wild-type strain. Hence, applying tried-and-tested technologies in genetic engineering with non-model organisms is the most promising route forward.
In 2018 three scientists received the Nobel Prize for their work on ‘directed evolution’, a variety of methods that can speed up and control the evolution of proteins to produce more useful and efficient molecules for use in technology and medicine. This technique opens up the possibility of improving the efficiency of proteins that produce metal nanoparticles.
The physicochemical properties of resulting biogenic nanoparticles can also be controlled to some extent. The pH, temperature and oxygen concentration in which bacteria is cultured can all influence biological metal precipitation. This means lithium-ion batteries can be ‘upcycled’ into nanoparticles for different applications. The recovered metals may even be regenerated back into battery material, creating a closed-loop recycling process where the raw material returns to its original place[3]. Alternatively, the nanoparticles can be exploited for their catalytic properties that add value to the recycling method.
The full cycle
It is essential to recognise that the success of the end product will be dependent on its economic and environmental value. The process behind upcycling lithium-ion batteries biologically must compete with chemical alternatives. Government policies – in the form of monetary awards or penalties – will also need to work alongside the technology to encourage industry in the right direction. Nonetheless, bacteria can be a way to secure a safe supply chain for metals. The exponentially increasing demand can be met and hazardous waste can be minimised. It takes us a step closer towards promoting a circular economy for lithium-ion batteries.
Giovanni Maddalena is a PhD researcher at the University of Edinburgh investigating the biosynthesis of metal nanoparticles in the Horsfall Lab. He is a member of the Faraday Institution’s ReLiB team focused on creating a circular economy for li-ion batteries.
Professor Louise Horsfall FRSB is chair of sustainable biotechnology at the University of Edinburgh, an EPSRC Fellow in engineering for sustainability and resilience, and a co-investigator on ReLiB, an interdisciplinary research project funded by UKRI through the Faraday Institution.
Dr Virginia Echavarri-Bravo is a Research Fellow at the University of Edinburgh working on the ReLiB project, developing biological processes for metal recycling
2) The Faraday Institution – ReLiB. Available from: www.faraday.ac.uk/research/lithium-ion/recycle-reuse
3) Kim, T. Y. et al. Biosynthesis of nanomaterials by Shewanella species for application in lithium-ion batteries. Front. Microbiol. 9, 2817 (2018).
4) Janssen, P. J. et al. The complete genome sequence of Cupriavidus metallidurans strain CH34, a master survivalist in harsh and anthropogenic environments. PLoS One 5(5), e10433 (2010).
5) Shi, L. et al. Respiration of metal (hydr)oxides by Shewanella and Geobacter: a key role for multihaem c-type cytochromes. Mol. Microbiol. 65(1), 12–20 (2007).
6) Wright, M. H. et al. Production of manganese oxide nanoparticles by Shewanella species. Appl. Environ. Microbiol. 82(17), 5402–5409 (2016).
7) Capeness, M. J. et al. Shotgun proteomic analysis of nanoparticle-synthesizing Desulfovibrio alaskensis in response to platinum and palladium. Microbiol. 165(12), p1282–1294 (2019).
8) Choi, Y. et al. Recombinant Escherichia coli as a biofactory for various single- and multi-element nanomaterials. Proc. Natl. Acad. Sci. 115(23), 5944–5949 (2018).
9) García-García, J. D. et al. Bio-recovery of non-essential heavy metals by intra- and extracellular mechanisms in free-living microorganisms. Biotechnol. Adv. 34(5), 859–873 (2016).
10) Bütof, L. et al. Synergistic gold–copper detoxification at the core of gold biomineralisation in Cupriavidus metallidurans. Metallomics 10(2), 278–286 (2018).